Mastering the Electron Microscope: A Complete Guide
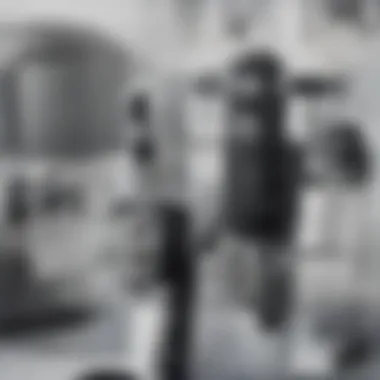
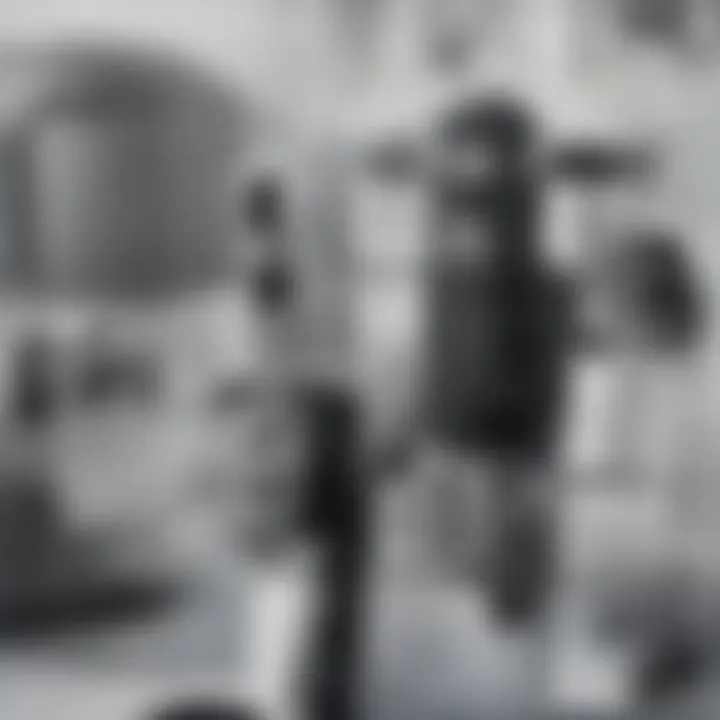
Intro
The use of electron microscopes represents a pivotal advancement in the field of microscopy, enabling researchers and scientists to explore the ultra-structure of materials at unprecedented resolutions. Unlike traditional light microscopes, electron microscopes utilize beams of electrons to achieve magnification levels that can vastly exceed optical limits. This article serves to guide users on effectively harnessing the capabilities of this sophisticated equipment, covering essential concepts, operational principles, image acquisition, and various applications across disciplines ranging from biology to materials science.
This guide is structured to accommodate a wide range of user experience levels, particularly targeting students, researchers, educators, and professionals who seek to deepen their understanding of electron microscopy. Through the following sections, readers will gain insights into critical terminology, outline methodologies, and investigate practical implications.
By engaging with this material, users will be better equipped to perform experiments, analyze results, and contribute to the advancing knowledge in the respective fields they inhabit.
Prolusion to Electron Microscopy
Electron microscopy is a crucial tool in modern scientific research. Its significance lies in its ability to offer high-resolution images of samples at the nanoscale. This capability allows researchers to study fine details that are often inaccessible using conventional optical microscopes. Understanding the fundamentals of electron microscopy is essential for students, researchers, and professionals looking to leverage this technology in their work.
Historical Development
The journey of electron microscopy began in the 1930s. Physicist Ernst Ruska, along with Max Knoll, developed the first electron microscope in 1931. The device utilized a beam of electrons instead of visible light, resulting in far superior resolution. This innovation paved the way for various advancements in microscopy techniques. Over the decades, electron microscopy has evolved, leading to the development of various types that cater to different scientific needs. Today, it plays a critical role in disciplines such as biology, materials science, and nanotechnology.
Types of Electron Microscopes
Electron microscopes can be classified into several types based on their operational principles and application scopes.
Scanning Electron Microscopes
Scanning Electron Microscopes (SEM) are widely used in research due to their excellent depth of field and three-dimensional imaging capabilities. They work by scanning a focused beam of electrons across the surface of a sample. This interaction generates various signals that provide information about the sampleβs composition and topography. The key characteristic of SEM is its ability to create high-resolution images without requiring the sample to be sliced into thin sections. This makes it particularly beneficial for examining the surface structure of larger specimens. However, SEM also has disadvantages, such as limited resolution compared to Transmission Electron Microscopes (TEM).
Transmission Electron Microscopes
Transmission Electron Microscopes (TEM) are another type of electron microscope, best known for their incredibly high resolution, which can reach atomic levels. TEM operates by transmitting electrons through a very thin sample. This technique allows scientists to observe internal structures in specimens, making it particularly useful for materials science and biological research. The main advantage of TEM is its unparalleled resolution, enabling detailed analysis of microstructures. Yet, the requirement of extremely thin sections can be a drawback, complicating the sample preparation process.
Environmental Electron Microscopes
Environmental Electron Microscopes (ESEM) represent a significant advancement in electron microscopy technology. They allow for the imaging of samples in their natural or hydrated states, providing insights into dynamic processes that occur in real time. ESEM is particularly valuable for biological and environmental studies where moisture and gas conditions are critical. One unique feature of ESEM is its capability to control environmental conditions within the microscope chamber. While this technology offers remarkable advantages, such as the ability to study live samples, it can be more complex and costly to operate compared to traditional techniques.
Understanding the different types of electron microscopes is vital for selecting the appropriate tool for specific research goals.
The exploration of these electron microscopy techniques lays the groundwork for appreciating their applications in various scientific fields.
Fundamentals of Electron Microscopy
Understanding the fundamentals of electron microscopy is crucial for anyone seeking to utilize this sophisticated tool effectively. Electron microscopy allows researchers to explore materials at an atomic and molecular level, providing insights that optical microscopy cannot achieve. It is important because it underlies the operational techniques, sample preparation, imaging methods, and ultimately the interpretation of results. Comprehensive knowledge in this area grants users the ability to maximize the potential of this advanced equipment, leading to more accurate and meaningful research outcomes.
Operational Principles
The operational principles of electron microscopy hinge on the interaction between electrons and the specimens being studied. Unlike light microscopes that rely on visible light, electron microscopes utilize a beam of electrons to illuminate the sample. This process allows for much greater magnification and resolution, often up to millions of times.
An essential aspect of these operational principles is the wave-particle duality of electrons, making them behave both as particles and waves. This characteristic enables them to resolve very fine details of a specimen, which is critical for applications in fields such as materials science and biology.
Key Components of an Electron Microscope
Key components in an electron microscope play a significant role in its function and capabilities. Each part contributes to the overall effectiveness of the microscope, ensuring high-quality imaging and analysis.
Electron Source
The electron source is vital in the production and maintenance of the electron beam. Various types of electron sources exist, such as thermoionic sources and field emission sources. The key characteristic of these sources lies in their ability to generate a stable and coherent beam of electrons.
The use of a field emission electron source is a beneficial choice for high-resolution applications due to its brightness and narrow energy spread. It allows for clearer images and better analytical capabilities. One distinct feature of this source is its reduced thermal noise compared to traditional sources, which leads to minimal sample damage and improved resolution.
Lenses and Magnification
Magnification and lenses are crucial for focusing the electron beam onto the specimen. Unlike optical lenses, which bend light, electron lenses manipulate electromagnetic fields to control the path of the electrons. This manipulation enables powerful magnification capabilities, essential for examining minute details.
A significant advantage of electron lenses is their ability to achieve much higher resolution than optical lenses. However, they require precise alignment and calibration, which can be a disadvantage during operation. The unique feature of these lenses is the capability to switch between different magnification levels seamlessly, accommodating various types of analyses and observations.
Detectors
Detectors play an important role in capturing and converting the electron signals into images. Common types of detectors used in electron microscopy include scintillator-based detectors and semiconductor detectors. Each type has its key characteristic, primarily its ability to convert electrons into a visible signal that can be captured for further analysis.
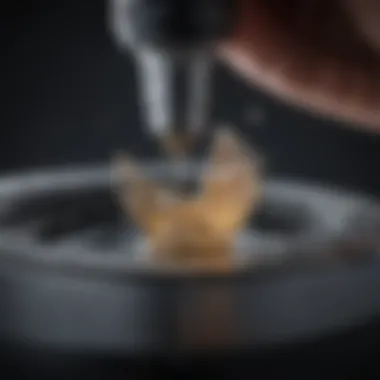
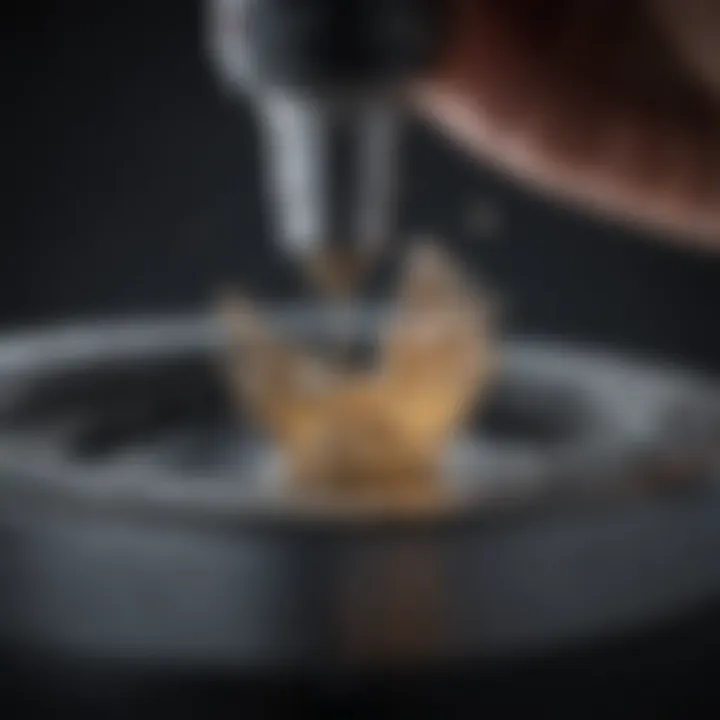
Scintillator detectors are often favored for their sensitivity and speed, making them a popular choice in high-throughput applications. Their unique feature lies in their ability to provide instant feedback, enabling quick adjustments during experiments. On the downside, they may have limits in terms of energy resolution compared to other detector types. By understanding the dynamics of detectors, users can make informed choices about which to implement in their studies.
Preparing Samples for Electron Microscopy
Preparing samples for electron microscopy is crucial for achieving reliable and high-quality results. In this section, various facets of sample preparation will be explored, addressing its significance, diverse types of samples, and the techniques involved.
Types of Samples
Biological Samples
Biological samples often come from tissues, cells, or microorganisms. Their importance lies in the detailed examination of structural organization and cellular interactions, contributing significantly to fields like medicine and biology. The key characteristic of biological samples is their complexity, providing rich information at high magnifications. This makes them a popular choice for electron microscopy as it allows researchers to visualize cellular structures not observable through other methods. However, they can also be delicate. The challenge lies in preserving the sample without altering its natural state, which can sometimes result in artifacts.
Materials Science Samples
Materials science samples encompass metals, polymers, and ceramics. The primary focus with these samples is on understanding material properties at a micro or nano scale. A principal characteristic of these samples is their physical and chemical properties, which can be analyzed to enhance material performance. They are favored for electron microscopy because the technique reveals details about grain structure and phase distributions. However, the preparation often requires careful techniques to avoid modifying physical properties, such as thermal or mechanical alterations during the process.
Nanoparticles
Nanoparticles have unique properties that differ from bulk materials due to their small size. They receive considerable attention in various fields, such as nanotechnology and medicine. A key trait of nanoparticles is their high surface area-to-volume ratio, which influences their behavior in applications. This makes them a beneficial choice for electron microscopy, as analysis can provide insights into their interactions and functionalities. On the downside, preparing nanoparticles for imaging can be more complex due to the need for precise control over their environment during preparation.
Sample Preparation Techniques
Fixation and Dehydration
Fixation and dehydration are essential steps in preserving biological samples. These processes help to stabilize the sample, preventing degradation and preserving cell structures. The primary characteristic of fixation is that it typically involves chemical agents that cross-link proteins and preserve cellular architecture. This is beneficial as it maintains the sample's integrity for imaging. However, different fixation methods can affect the suitability of the sample for electron microscopy, leading to variances in imaging quality. Additionally, the dehydration process can lead to shrinkage, which must be carefully managed.
Embedding
Embedding further supports sample integrity, allowing for better handling during sectioning. This involves surrounding the sample in a solid medium, which aids in cutting thin sections without damaging the specimen. The key characteristic of embedding materials is that they can provide structural stability. This technique is popular because it allows very thin sections to be cut, which facilitates high-resolution imaging. Nevertheless, embedding materials can sometimes obscure details if not chosen correctly, and thus require careful selection based on the sample type.
Sectioning and Staining
Sectioning and staining involve cutting the embedded sample into thin slices for electron microscopy analysis. This step is vital for revealing features at the cellular level. A fundamental characteristic of this process is that proper sectioning creates uniform slices that enhance image quality. Staining can help differentiate between structures based on electron density. This process is beneficial since it allows researchers to highlight specific components within the sample, aiding in the interpretation of complex structures. However, over-staining or poorly sectioned samples can lead to misinterpretation of structures and incomplete data.
In essence, careful sample preparation is a cornerstone of effective electron microscopy, influencing the quality and accuracy of the data obtained.
Ultimately, understanding these aspects of sample preparation will facilitate better outcomes in electron microscopy, leading to more accurate observations and interpretations.
Operating the Electron Microscope
Operating an electron microscope is a crucial phase in utilizing this advanced analytical tool. Understanding how to effectively operate the microscope is essential for obtaining high-quality images and data. This section focuses on specific processes and methodologies that enhance the userβs ability to extract meaningful information from their samples. The procedures for setup, calibration, and imaging techniques will be elaborated upon, as they are central to achieving reliable results in scientific investigations.
Setting Up the Microscope
Setting up the electron microscope is the first step to successful imaging. This involves several preparatory tasks that are vital to ensure that the instrument operates correctly and provides accurate data. Proper setup minimizes the risk of errors and sample damage.
Calibration Procedures
Calibration procedures are fundamental to the operation of an electron microscope. They ensure that the imaging system is functioning as intended. This process involves adjusting the microscope's parameters to match a known standard, allowing for precise measurement and imaging.
Key characteristics of calibration include its systematic approach. It is a beneficial practice because it helps in establishing accuracy and reliability in results. The unique feature of these procedures is their role in maintaining the microscope's performance over time. Regular calibration can help detect deviations in imaging quality before they become significant issues, thus ensuring consistency in research outcomes.
However, there can be disadvantages. Calibration processes require time and skilled personnel who understand the specific requirements of each instrument model. Missteps during calibration may lead to incorrect imaging results, thus impacting the overall research quality.
Aligning the Beam
Aligning the beam is another critical aspect of preparing the microscope for operation. This process involves adjusting the electron beam to ensure it is centered and properly directed. Proper beam alignment is essential for optimal imaging and accurate data collection.
The key characteristic of beam alignment is its direct impact on resolution and contrast in the obtained images. It is a popular practice within electron microscopy because correct alignment can significantly enhance the visibility of microstructures. The unique feature of aligning the beam is its need for meticulous adjustments, including focusing lenses and minimizing astigmatism.
The advantages of successful beam alignment include improved image clarity and detail. However, it can also present challenges, especially for new users who may struggle with making the necessary adjustments. Experience is often needed to align the beam effectively.
Imaging Techniques
Imaging techniques play a significant role in how data is visualized and interpreted in electron microscopy. Appropriate imaging methods contribute to obtaining high-quality images and can greatly affect analysis outcomes.
Low-Voltage Imaging
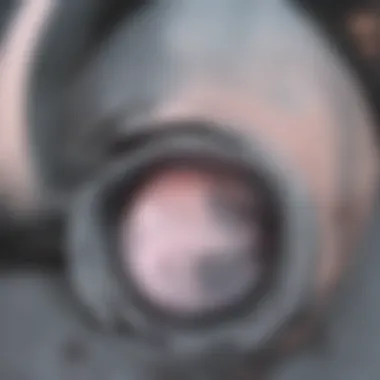
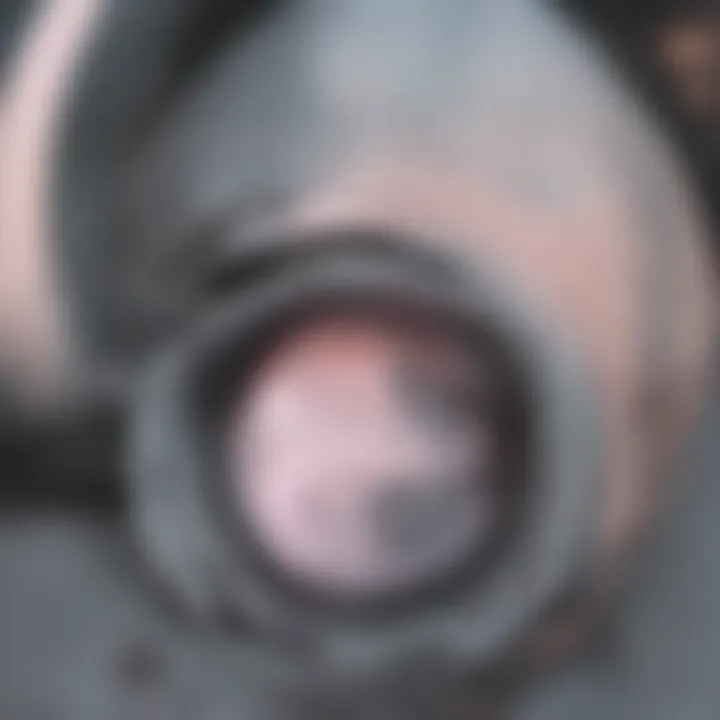
Low-voltage imaging is a technique used to minimize sample damage while achieving clear images. This method involves operating the microscope at lower accelerating voltages, which reduces the energy of electrons hitting the sample.
The key characteristic of low-voltage imaging is its ability to enhance contrast, particularly for biological samples. This is a beneficial choice when working with delicate samples that may be prone to damage under higher voltages. The unique advantage of this technique is its potential to provide better preservation of sample integrity. However, one downside is that lower voltages can sometimes result in reduced resolution compared to higher voltage methods.
High-Resolution Imaging
High-resolution imaging is essential for applications that demand detailed structural information. This technique uses higher accelerating voltages and sophisticated optics to achieve images with fine detail.
The key characteristic of high-resolution imaging is its ability to reveal substructures within materials or biological samples. This is beneficial for materials science research where detailed analysis of microstructures is essential. The unique feature is its capacity to provide insights into material properties at the atomic level. Nevertheless, the trade-off involves an increased risk of damaging sensitive samples due to higher electron energies.
Three-Dimensional Imaging
Three-dimensional imaging is an advanced technique that allows researchers to visualize samples in a more comprehensive manner. It involves the use of specialized data acquisition methods to create 3D reconstructions from multiple 2D images.
The key characteristic of three-dimensional imaging is its ability to present complex spatial relationships within a sample. This is a beneficial approach for studies in fields such as nanotechnology, where understanding the structure in three dimensions can yield significant insights. The unique advantage of this technique is its overall representation of the sample architecture. However, it requires more advanced software and capabilities, which can complicate the imaging process and increase operational costs.
"Understanding the capabilities and techniques of electron microscopy is crucial for any researcher seeking to harness its potential effectively."
In summary, operating the electron microscope involves a series of steps that are foundational to effective imaging and data collection. Each aspect, from calibration to imaging techniques, contributes to the quality of the research performed. With careful attention to these critical processes, users can enhance their proficiency and reliability in utilizing electron microscopes for various scientific investigations.
Data Analysis and Interpretation
Data analysis and interpretation represent a pivotal component in utilizing an electron microscope. The information drawn from experimentation can significantly influence research outcomes. Understanding how to analyze imagery and data effectively ensures that conclusions drawn are reliable and meaningful. Key considerations in this area include the various techniques available for image processing and the nature of data interpretation, which varies based on the objectives of the study.
Image Processing Techniques
Software for Image Analysis
The selection of software for image analysis greatly affects the data interpretation process. Options such as ImageJ and Fiji stand out due to their user-friendly nature and comprehensive range of tools for processing images obtained from electron microscopes. A crucial aspect of such software is their ability to handle large datasets efficiently. This efficiency is vital given the volume of information produced through electron microscopy.
Key features of software like ImageJ include customizable plugins and a robust community support system. This makes it favorable for many researchers and students. Additionally, these programs allow for advanced processing features such as deconvolution and image stacking. Yet, novice users might find the initial learning curve challenging, necessitating time spent in training.
Quantitative vs. Qualitative Analysis
When discussing quantitative versus qualitative analysis, it is essential to understand how these methodologies contribute to meaningful data interpretation. Quantitative analysis focuses on numerical data, allowing for statistical comparisons and measurements of microstructures such as particle size and distribution. This approach is often favored when precision is paramount.
On the other hand, qualitative analysis offers insights into properties and behaviors of samples without relying purely on numeral outputs. This method aids in understanding the significance of the structures observed. A unique aspect of qualitative analysis is its ability to provide context and narrative to data, which is often overlooked in strictly quantitative approaches. Both methods have their advantages and limitations; the choice depends on the study's goals and framework.
Interpreting Results
Identifying Microstructures
Identifying microstructures plays a critical role in the overall analysis of results. It helps in understanding the relationship between structure and properties of materials. Microstructures can reveal defects, grain boundaries, and phases in materials which are essential in fields ranging from materials science to biology.
The capability to accurately identify these structures is essential in fields such as nanotechnology where the performance of materials often depends on their microstructural characteristics. Knowledge of specific identification techniques can enhance research relevance. However, this process can require experienced judgment and knowledge of the subject matter to avoid misinterpretation of results.
Assessing Material Properties
Assessing material properties through electron microscopy enables researchers to make informed decisions regarding the suitability of materials for specific applications. The technique can reveal important mechanical, thermal, and electrical properties derived from the microstructural cues noted in the imagery.
Notably, the distinctive advantage of using electron microscopy lies in its ability to offer high-resolution insights that are not always attainable through other traditional methods. Nevertheless, it is essential for users to recognize that while electron microscopy provides valuable data, it should not replace other characterization methods but rather complement them to garner a complete assessment.
Applications of Electron Microscopy
The applications of electron microscopy are vast and critical across various fields. The technology enhances clarity and detail when observing materials at the nanometer scale. Understanding these applications is key for researchers and professionals who seek to utilize the potential of electron microscopes in their work. Each application serves specific needs, ranging from detailed biological studies to advanced materials science investigations.
Biomedical Research
Electron microscopy plays a pivotal role in biomedical research, especially in the analysis of cellular structures. The high-resolution capabilities allow scientists to visualize intricate details of cell organelles, viruses, and tissues. Surfacing the fine structures enhances the understanding of disease mechanisms and cellular interactions.
In studying infectious diseases, electron microscopes enable the detailed observation of pathogens, which is crucial for vaccine development and infectious disease control. Moreover, researchers can examine the changes in cellular morphology due to diseases, which can aid in early diagnosis.
Additionally, advances in techniques like cryo-electron microscopy allow the observation of biomolecules in near-native states, providing insights into protein structures and functions. This information is valuable in drug design, offering translations from structural biology to therapeutic applications.
Nanotechnology
Nanotechnology benefits significantly from the use of electron microscopy. As nanomaterials become integral to technological advancements, electron microscopes provide critical insights into their properties and behaviors at the nanoscale. Characterizing nanostructures helps understand their mechanical, electrical, and optical properties, which is vital for innovation.
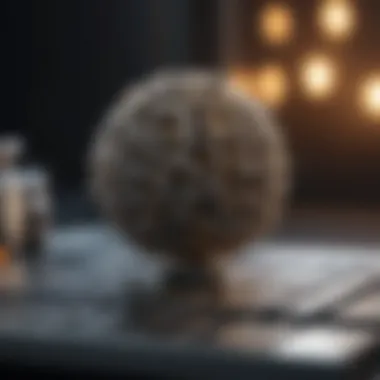
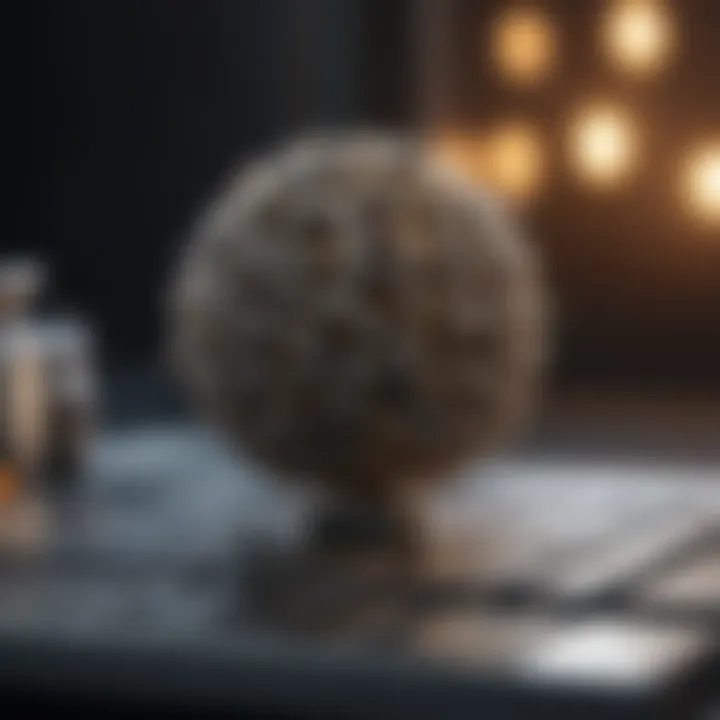
The ability to manipulate materials at the atomic level using electron microscopy further facilitates the design of novel materials. Applications in fields like electronics, energy storage, and pharmaceuticals rely heavily on the understanding provided by this microscopy technique. By investigating the very small, scientists can design safer and more effective products.
Materials Science
In materials science, electron microscopy is indispensable for analyzing microstructures. Understanding the composition and arrangement of materials contributes to developing stronger, lighter, and more durable substances, which is particularly important in industries such as aerospace and automotive.
Electron microscopes assist in failure analysis, offering insights into fracture surfaces and defects in materials. This knowledge is essential for improving materials engineering and ensuring safety in applications.
Moreover, advanced imaging techniques allow scientists to study materials under various conditions, helping to comprehend phase changes and mechanical properties. This data can ultimately lead to innovation in materials usage across different sectors.
The ability to view materials at the atomic level transforms research across many scientific disciplines.
Challenges and Limitations
The field of electron microscopy offers remarkable imaging capabilities, yet it does not come without its challenges and limitations. Understanding these aspects is vital for users looking to maximize the efficiency and effectiveness of their research. This section will explore both technical and user challenges that can affect the use of electron microscopes.
Technical Challenges
Sample Damage
Sample damage is one of the most significant challenges faced during electron microscopy. This phenomenon occurs due to the interaction between the electron beam and the sample. High-energy electrons can cause structural changes or even complete destruction of biological or sensitive materials. This factor makes it crucial for researchers to carefully consider the nature of their samples.
The key characteristic of sample damage lies in its unpredictability; different materials react differently to electron irradiation. For instance, biological tissues are often more susceptible to radiation damage than inorganic samples. This is a particularly relevant issue for studies that involve sensitive biological samples. One beneficial aspect of understanding sample damage is that it pushes researchers to adopt more refined techniques and preparation methods to minimize negative impacts. However, it remains a significant hurdle that can compromise the reliability of results.
Resolution Limits
Resolution limits refer to the maximum level of detail that can be captured by an electron microscope. Even though electron microscopes can achieve extremely high resolutions, factors such as sample thickness and imaging conditions can reduce effective resolution. This has a direct impact on what can be visualized and analyzed.
The key characteristic of resolution limits is that they can restrict observations to larger structures, leading to potential overlooks of important details found at a nanoscopic level. Effective resolution can compromise data accuracy, a situation that researchers aim to avoid. Hence, the challenge lies not just in achieving high resolution, but also in maintaining it under varying experimental conditions. Understanding these limits can lead to improved methodology, although it can also prove to be a frustrating limitation in practice.
User Challenges
Skill Development
Skill development is an essential aspect when utilizing electron microscopes. Users must understand both the theoretical principles and practical applications, which requires a steep learning curve. This can be particularly challenging for novice users who may lack prior experience.
One key characteristic of skill development is the necessity of extensive training, which includes both hands-on experience and a solid grasp of the underlying technology. This demand for proficiency can be both beneficial and daunting for institutions aiming to incorporate this technology effectively. Moreover, the depth of knowledge required for advanced techniques may create barriers for users who are not adequately supported through ongoing training programs. The advantage of overcoming these challenges, however, fosters a highly skilled workforce capable of extracting valuable insights from electron microscopy.
Operational Costs
Operational costs can pose a significant barrier to the practical use of electron microscopes. These costs arise from maintaining advanced equipment, ensuring proper sample preparation, and ongoing operational expenses such as labor and supplies. Institutions that aim to employ these tools must make careful budget considerations.
The key characteristic of operational costs is their dual nature: while they represent a considerable investment, they can also lead to substantial returns in research output and innovation. It is beneficial for institutions to weigh the costs against the potential scientific advancements offered by electron microscopy. However, this financial burden can limit accessibility to only well-funded laboratories, thereby constraining opportunities for broader research applications.
In summary, addressing the challenges and limitations of electron microscopy is essential for maximizing its application across various fields. By understanding the nuances of sample damage, resolution limits, skill development, and operational costs, researchers can better navigate the complexities of this powerful tool.
Through keen attention to these challenges, users can improve their proficiency and ultimately enhance the reliability and quality of their research outcomes.
Future Directions in Electron Microscopy
The field of electron microscopy is poised for significant advancements. Future directions in electron microscopy not only reflect the technological evolution but also focus on increasing accuracy and efficiency in various scientific fields. Understanding these potential pathways is vital for researchers and technologists as they adapt to new methodologies in imaging and analysis. The exploration of future directions will likely yield enhanced techniques that can overcome current limitations, ultimately broadening the scope of electron microscopy applications.
Technological Innovations
Technological innovations are the cornerstone of progress in electron microscopy. New equipment developments, such as improved electron sources and detectors, are critical. These innovations can significantly impact resolution and imaging speed, making the process more efficient.
One notable innovation includes:
- Cryo-Electron Microscopy (Cryo-EM): This method preserves samples in a frozen state, enabling structural biology studies at near-native conditions. It permits visualization of biomolecules without the need for staining, hence reducing artifacts.
- Spherical Aberration-Corrected Microscopes: These instruments enhance imaging by correcting spherical aberrations, resulting in sharper images and higher resolution. This technology is particularly beneficial for studying nanoscale materials.
"The rapid advancements in detector technology allow for faster data collection while enhancing the signal-to-noise ratio, ultimately improving the quality of the images captured."
These innovations not only push the boundaries of what can be visualized but also reduce user skill dependencies, making the technology more accessible.
Potential Research Frontiers
Potential research frontiers in electron microscopy are vast. The developments in imaging capabilities lead to new possibilities in various scientific domains. Researchers are particularly interested in merging electron microscopy with other techniques such as X-ray tomography and mass spectrometry. Such interdisciplinary approaches enable more comprehensive studies.
Some promising areas include:
- Nanotechnology: As the field evolves, electron microscopy will be crucial for characterizing nanomaterials with precision. Understanding the interactions at this scale could lead to breakthroughs in material science.
- Biomolecular Imaging: With advancements in cryo-EM, researchers can explore the dynamics of complex biological systems in unprecedented detail. This is vital for drug design and understanding cellular mechanisms.
These frontiers not only enhance the scientific community's understanding but also pave the way for novel applications across various disciplines, enhancing the role of electron microscopy in research.