Optimizing PCR Techniques: Key Approaches and Factors
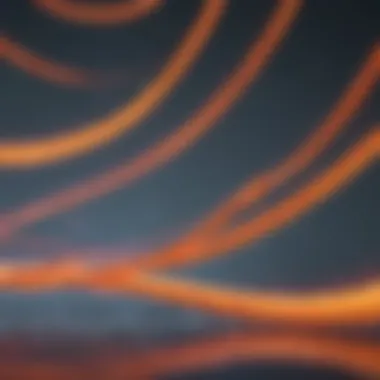
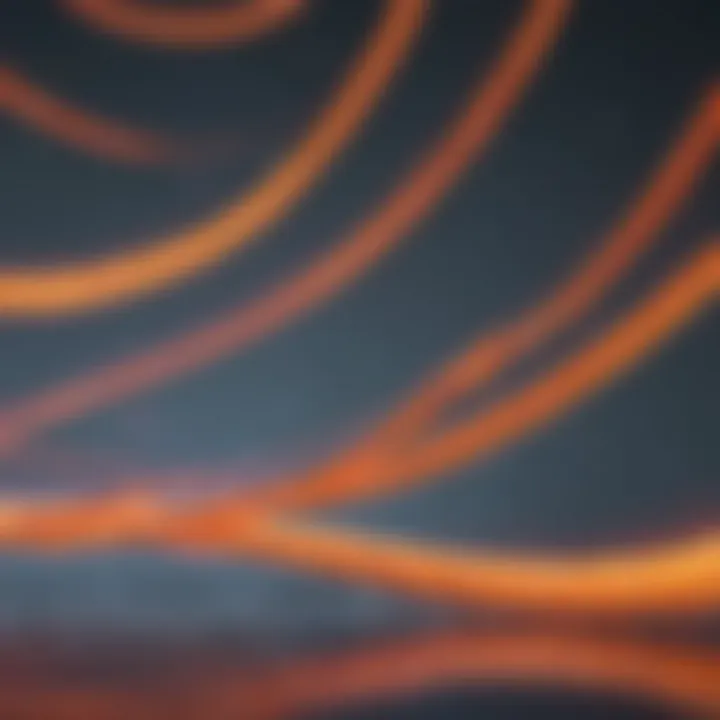
Intro
Polymerase Chain Reaction (PCR) has become a cornerstone of molecular biology, enabling significant advancements in genetic research, diagnostics, and biotechnology. However, achieving optimal results from PCR requires not only understanding the fundamental processes involved but also careful consideration of various parameters that can impact amplification efficiency. This exploration of PCR optimization provides insights for students, researchers, educators, and professionals alike, all of whom seek to enhance the precision and reliability of their molecular experiments.
Key Concepts and Terminology
Definition of Key Terms
- Polymerase Chain Reaction (PCR): A robust method used for amplifying segments of DNA, making it possible to generate millions of copies from a small sample.
- Primer: Short sequences of nucleotides that initiate DNA synthesis during the PCR process, specific to the target DNA sequence.
- Thermal Cycling: The repeated heating and cooling phases in PCR that allow denaturation, annealing, and extension to occur.
- Enzyme: A biological catalyst, such as Taq polymerase, that facilitates the synthesis of new DNA strands.
- Contaminants: Unwanted substances within the PCR reaction that can inhibit amplification and lead to unreliable results.
Concepts Explored in the Article
This article will systematically cover essential components of PCR optimization, including:
- Thermal Cycling Conditions: Understanding the significance of temperature settings and timing in the amplification process.
- Primer Design Strategies: Evaluating the role of primer specificity and concentration to enhance target binding.
- Enzyme Selection: Comparing various DNA polymerases and their properties to determine optimal performance in different conditions.
- Impact of Contaminants: Identifying common contaminants that can affect PCR efficiency and how to mitigate their effects.
Findings and Discussion
Main Findings
Through meticulous analysis of current research and best practices, the optimization of PCR processes reveals several trends:
- Precision in Temperature Settings: Fine-tuning denaturation, annealing, and extension temperatures can lead to significant improvements in yield and specificity.
- Shorter Primer Lengths: Employing shorter primers can reduce non-specific binding while maintaining effective amplification.
- Choice of Polymerase: The selection of the polymerase influences fidelity and amplification speed; for instance, high-fidelity enzymes are preferable for applications requiring accuracy.
Potential Areas for Future Research
Future studies can focus on:
- Developing advanced algorithms for automated primer design.
- Exploring the effects of new heat-stable enzymes under diverse reaction conditions.
- Investigating the long-term stability of reactions in the presence of known contaminants.
"Optimizing PCR is not merely a technical task, but an art that requires profound understanding of biological principles."
Preamble to PCR
Polymerase Chain Reaction (PCR) is a revolutionary technique that enables the amplification of specific DNA sequences. This section provides foundational knowledge essential to understanding the broader topic of PCR optimization. By delving into the historical context and fundamental principles of PCR, readers gain insights into why optimizing this technique is not just advisable but crucial for achieving reliable and reproducible results.
Historical Context
The inception of PCR took place in the early 1980s, credited to Kary Mullis. His work was pivotal in enabling scientists to replicate DNA quickly and efficiently, a task that was once time-consuming and technically challenging. Initially, PCR was a laboratory curiosity, yet it soon became indispensable in various fields including forensics, genetics, and medical diagnostics. With the method established, a myriad of applications emerged, allowing for genetic testing, cloning, and the development of genetically modified organisms. Understanding this historical backdrop provides a context for contemporary improvement efforts in PCR methodologies.
Fundamental Principles of PCR
At its core, PCR relies on three essential steps: denaturation, annealing, and extension.
- Denaturation: This step involves heating the DNA sample to around 94-98 °C. High temperatures break the hydrogen bonds between the DNA strands, resulting in two single strands.
- Annealing: Following denaturation, the temperature is lowered to about 50-65 °C, allowing primers to bind to the complementary sequences on the single-stranded DNA. The choice of primers is crucial, as they dictate the specificity of the amplification.
- Extension: In this phase, the temperature is usually adjusted to about 72 °C. DNA polymerase synthesizes new DNA strands by incorporating nucleotides complementary to the target sequence.
These repeated cycles effectively double the amount of targeted DNA with each cycle, creating an exponential increase in the DNA quantity, which could then be analyzed further.
PCR stands as a cornerstone of genetic research, embodying both analytical power and the need for precise optimization within its applications.
Importance of Optimization in PCR
Optimization in Polymerase Chain Reaction (PCR) is crucial for achieving reliable and reproducible results in DNA amplification. The ability to fine-tune various parameters means that researchers can obtain higher yields of the target DNA, reduce non-specific amplification, and improve overall reaction efficiency. Each element of the PCR process significantly affects the final output, making optimization not just a beneficial practice but a necessary one in many instances.
The main aspects to focus on during optimization include the quality and quantity of the DNA template, primer design, enzyme selection, buffer composition, and thermal cycling conditions. Each factor contributes uniquely to the success of the PCR reaction:
- Consistent Results: Optimized protocols lead to consistent amplification results, reducing variability across experiments.
- Specificity: By carefully designing primers and adjusting their concentrations, researchers can minimize non-specific binding and amplification.
- Time and Resource Efficiency: Efficient PCR reduces the need for repeated trials, saving time and resources.
Ultimately, the goal of optimization is to achieve a balance where all parameters work harmoniously together. This not only maximizes the sensitivity of the assay but also minimizes the likelihood of contamination, which can lead to erroneous conclusions.
Impact of Optimized Protocols
The implementation of optimized PCR protocols can greatly enhance the outcomes of molecular biology projects. When protocols are carefully adjusted, results become more predictable and reliable. For example, fine-tuning the annealing temperature can increase specificity, allowing for cleaner amplification of the desired product.
In practical settings, this leads to several beneficial outcomes:
- Enhanced Sensitivity: Lower limits of detection can be achieved, which is particularly important in applications such as clinical diagnostics.
- Increased Reproducibility: Well-optimized protocols allow other researchers to replicate results accurately, fostering collaboration and progression in scientific research.
- Broader Applications: Optimization makes it feasible to apply PCR across diverse types of samples, including those found in forensics, environmental studies, and clinical diagnostics.
Challenges in PCR Reactions
Despite its powerful applications, PCR still poses challenges that can hinder optimal performance. Common issues include:
- Contamination: This is a persistent problem that can introduce foreign DNA, compromising results.
- Inhibition: Certain substances present in the sample, such as proteins or phenolic compounds, can inhibit the reaction.
- Temperature Variability: Inconsistent thermal profiles can lead to inefficient amplification.
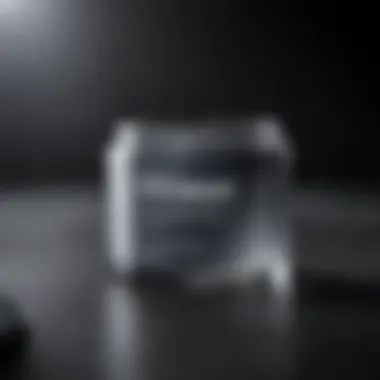
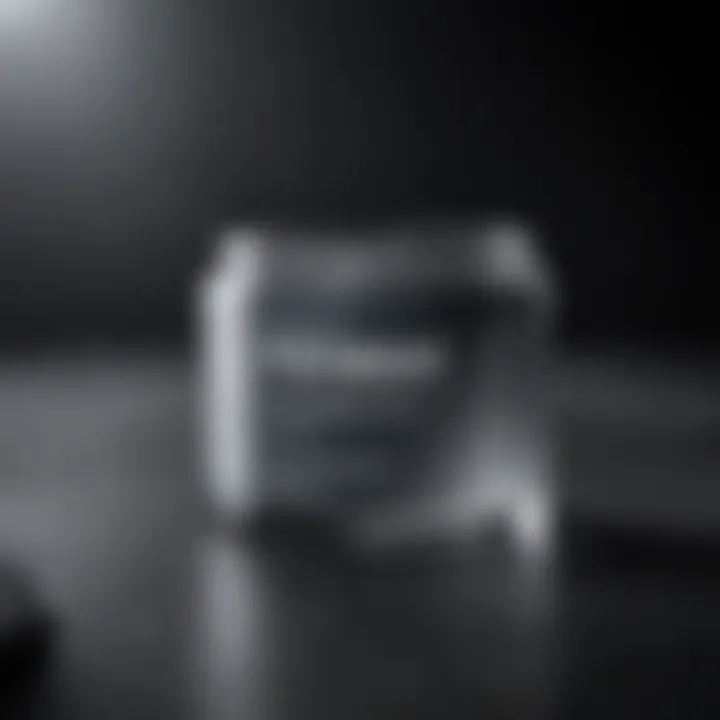
Recognizing and addressing these challenges is a crucial part of the optimization process. Identifying sources of contamination and employing measures to minimize their impact can profoundly influence the outcome. Likewise, selecting appropriate conditions tailored to the specific sample can reduce the effects of inhibitors.
"The journey of optimization is continuous; even small adjustments can lead to significant improvements in PCR results."
In summary, the importance of optimization in PCR cannot be overemphasized. It forms the bedrock of achieving reliable and reproducible results, essential in advancing both scientific knowledge and practical applications.
Key Parameters in PCR Optimization
The optimization of Polymerase Chain Reaction (PCR) is a complex process that relies on several key parameters. Each of these parameters can affect the efficiency and accuracy of DNA amplification. Understanding these factors is essential for any practitioner aiming to achieve high-quality results. A detailed grasp of these key elements not only optimizes the PCR reactions but also meets the specific requirements of different applications.
DNA Template Quality and Quantity
The quality and quantity of the DNA template significantly impact the success of PCR. Contamination, degradation, or improper concentrations can lead to suboptimal results. High-quality DNA is necessary for reliable amplification. Contaminants such as proteins, phenols, or salts may interfere with the reaction, causing issues like non-specific amplifications or low yields. As for quantity, having an appropriate amount of DNA template is crucial. Too little template may result in failed amplification, while too much can inhibit the reaction. Generally, a template concentration ranging from 1 to 10 ng per reaction is recommended for optimal results.
Primer Design Considerations
Primer design is another crucial factor in PCR optimization. Primers must be specific to the target sequence, as non-specific binding can lead to amplification of unintended products. Their melting temperature (Tm) should also be taken into account; mismatched Tm values can cause problems during annealing. Ideally, the Tm of both primers should be within a few degrees of each other. Additionally, primer length typically ranges from 18 to 25 nucleotides, as longer primers tend to increase specificity. Other considerations include avoiding repetitive sequences or secondary structures, which can further complicate amplification.
Enzyme Selection
The choice of DNA polymerase is equally important in PCR optimization. Different DNA polymerases function under varying conditions and have distinct properties. For example, Taq Polymerase is commonly used due to its heat stability but lacks proofreading capability. In contrast, high-fidelity enzymes like Phusion provide greater accuracy but may require specific cycling conditions. Selecting the right enzyme can directly affect amplification efficiency, specificity, and the overall yield of the desired product. Therefore, careful consideration of enzyme characteristics and their compatibility with other reaction components is necessary.
Buffer Components and Ion Concentration
Buffers are essential to maintain optimal pH and ionic strength during PCR. Each buffer component plays a role in stabilizing the reaction environment. Typical components include Tris-HCl, KCl, and MgCl2. Magnesium ions, particularly Mg2+, are critical as they act as cofactors for DNA polymerase. The concentration of magnesium can significantly influence enzyme activity and thus affects the amplification process. A generally accepted magnesium concentration range is between 1.5 to 3 mM. Adjusting these factors can yield improved results, helping to optimize specificity and overall reaction efficiency.
Thermal Cycling Parameters
Lastly, thermal cycling parameters are vital to PCR optimization. This includes optimizing denaturation, annealing, and extension times as well as temperatures. Denaturation typically occurs at around 94 to 98°C, sufficient for template strand separation. Annealing temperatures should align with the Tm of primers for effective binding. Extension is usually set at 72°C, an effective working temperature for most polymerases. The duration of each step can also be tweaked; longer extension times may be needed for longer DNA products. Adjusting these thermal cycling conditions can hugely impact the yield and specificity of the PCR products.
Key Takeaway: A thorough understanding of these key parameters is essential for optimizing PCR processes to achieve desired outcomes. Attention to detail in each factor—DNA quality, primer design, enzyme choice, buffer concentrations, and thermal cycling—can significantly enhance the overall performance of PCR.
Thermal Cycling Optimization Techniques
Thermal cycling is a critical component in the Polymerase Chain Reaction (PCR) process. It refers to the series of temperature changes that facilitate the amplification of specific DNA sequences. Proper optimization of these thermal cycling parameters is essential to increase the efficacy and specificity of PCR, which ultimately influences the success of the experiment.
Adjusting Denaturation Temperature
Denaturation is the initial step in the thermal cycling process where the double-stranded DNA separates into single strands. This is typically achieved by heating the reaction mixture to a temperature ranging from 90°C to 98°C. The correct temperature is crucial, as too low can lead to incomplete melting while too high can cause damage to the DNA template and enzymes.
A commonly used approach for optimizing denaturation temperature involves a gradient PCR. This allows researchers to assess the impact of different temperatures on the reaction in a single run. Additionally, using high-fidelity DNA polymerases can enhance results at slightly lower temperatures. This necessity not only improves yield but also decreases the likelihood of non-specific amplification.
Optimizing Annealing Temperature
The annealing step is where primers bind to the single-stranded DNA templates. The annealing temperature is determined primarily by the melting temperatures of the primers used. If the temperature is too high, the primers may not bind to the template, resulting in low amplification. Conversely, if the temperature is too low, non-specific binding may occur, leading to unwanted products.
To properly optimize this temperature, performing a two-step or three-step PCR can be beneficial. In a two-step PCR, the annealing and extension steps are combined. In a three-step PCR, one can test a range of annealing temperatures to see which leads to the best specificity and yield. Adjusting the magnesium concentration can also impact primer binding efficiency, thus influencing the optimal annealing temperature.
Extension Time Considerations
During the extension phase, the DNA polymerase synthesizes new DNA strands from the template. The extension time required generally depends on the length of the DNA to be amplified and the velocity of the particular enzyme used. Shorter DNA products may require only a few seconds, while longer fragments may necessitate extended periods to achieve full extension.
Utilizing high-performance DNA polymerases that exhibit rapid extension rates can help in reducing the necessary time without compromising the accuracy of the product. However, careful monitoring of the outcomes is crucial since inadequate extension can result in shorter fragments or incomplete products.
"Optimizing thermal cycling parameters can lead to significant improvements in the yield and specificity of PCR reactions, which is essential for credible scientific results."
Engaging in trial and error with these critical temperature adjustments can lead to better understanding and mastery over PCR techniques, ultimately enhancing experimental reliability.
Strategies for Primer Optimization
The success of the Polymerase Chain Reaction (PCR) largely depends on the design and optimization of primers. Primers are short sequences of nucleotides that initiate the DNA synthesis process. Optimized primer design enhances annealing, specificity, and ultimately increases yield in PCR reactions. With inefficient primers, the process can produce nonspecific amplification or fail entirely. Thus, optimizing primers is an essential part of refining PCR protocols for accurate DNA amplification.
Design Software and Tools
In the modern landscape of molecular biology, the availability of various design software has transformed the way researchers develop primers. Several programs are specifically built to assist in primer design, ensuring optimal specificity and efficiency.
Popular tools include:
- Primer3: This widely used tool allows researchers to input specific parameters, such as the desired melting temperature (Tm), product size, and GC content, to generate suitable primers.
- NCBI Primer-BLAST: This combines primer design with a blast search, ensuring that primers are specific to the target sequence while also avoiding complementary sequences and potential secondary structures.
- OligoCalc: A web-based tool for calculating the properties of oligonucleotides. It provides information regarding self-dimerization and Tm values, crucial for primer design.
Using these tools, researchers can systematically create primers that meet the essential criteria for their PCR experiments. By inputing specific parameters, one can predict how well the primers will bind to the template DNA and minimize andineffect_ve amplifications.
Testing Primer Specificity
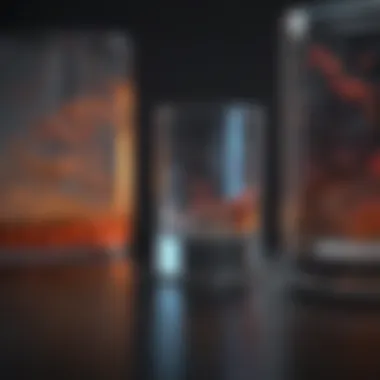
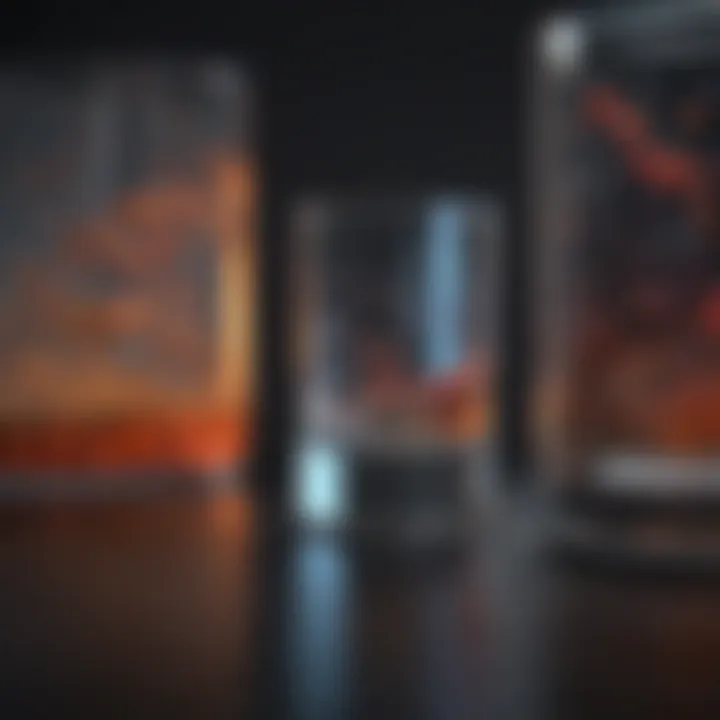
Testing for primer specificity is a fundamental step in PCR optimization. Specific primers bind only to the intended DNA sequences, directing the PCR process accurately. Non-specific binding can lead to unwanted products, complicating analysis and interpretation.
There are several methods to evaluate primer specificity:
- Melting Temperature (Tm) Assessment: Comparing the Tm of the primers against the template DNA helps to ensure they will anneal under the selected conditions.
- In Silico Analysis: Tools like NCBI BLAST can be employed to run a database search of the primer sequences against known genetic sequences. This validates that primers do not anneal with unintended targets.
- Gel Electrophoresis: After PCR amplification, analyzing the products through gel electrophoresis can reveal if the expected band is present. The presence of multiple bands can indicate non-specific binding.
- Sequencing: Sequencing of PCR products confirms that the desired amplicon has been produced without unintended mutations or amplification from unrelated sequences.
Effective primer optimization leads to more accurate amplifications, increasing the reliability of downstream applications such as cloning, sequencing, and quantitative PCR.
In summary, the strategies for primer optimization are essential for enhancing the effectiveness of PCR. Utilizing appropriate design software while rigorously testing primer specificity ensures that researchers can achieve reproducible and reliable results, thereby advancing their investigations in molecular biology.
Role of Enzymes in PCR
The role of enzymes in Polymerase Chain Reaction (PCR) is a critical factor that determines the efficiency and specificity of DNA amplification. Enzymes, particularly DNA polymerases, are the workhorses of the PCR process. Their main job is to replicate DNA by synthesizing new strands complementary to the template strand. Understanding the specific attributes and functionality of these enzymes helps researchers select the right polymerase for their experiments. This selection influences not just the yield of the product but also its accuracy and fidelity.
Types of DNA Polymerases
Several types of DNA polymerases are commonly used in PCR, each with distinct properties that cater to specific applications:
- Taq Polymerase: This is the most widely used enzyme due to its robustness and ability to withstand high temperatures during denaturation. It elongates the DNA chain rapidly, making it ideal for conventional PCR.
- Pfu Polymerase: Known for its high fidelity, Pfu polymerase has a proofreading ability that corrects errors during DNA synthesis. It is suitable for applications requiring high accuracy, such as cloning and mutagenesis.
- Q5 DNA Polymerase: This enzyme offers a balance between speed and fidelity, making it a good choice for complex templates or long PCRs. Its performance often exceeds that of both Taq and Pfu, and it is increasingly becoming a standard in labs.
- Bst Polymerase: This enzyme is employed primarily in isothermal amplification methods such as LAMP. Its activity at a constant temperature eliminates the need for thermal cycling, broadening the PCR applications.
The choice of DNA polymerase can significantly impact the results of an experiment, making it vital for practitioners to select the appropriate enzyme based on the specific needs of their assays.
Enzyme Concentration Optimization
Optimizing enzyme concentration is crucial in achieving the best results in PCR. An insufficient amount of enzyme may lead to incomplete amplification, whereas excessive concentrations can lead to non-specific products or inhibitors. The following considerations can guide practitioners in the optimization process:
- Empirical Testing: Start with a standard concentration, often recommended in the enzyme manual, and adjust as needed based on results. Typical concentrations range from 0.5 to 2.5 units per 50 µL reaction.
- Template Complexity: For complex templates, a higher concentration of enzyme may be necessary. Conversely, simple templates may yield satisfactory results with less enzyme.
- Amplification Efficiency: Performing a series of reactions with differing enzyme concentrations allows one to identify the optimal amount that yields the best amplification efficiency with high specificity.
- Reaction Conditions: Factors such as buffer composition, ion concentration, and the presence of PCR inhibitors can also affect the optimal enzyme concentration. Adjusting these variables in tandem with enzyme concentration can provide better results.
"Enzyme concentration is a critical variable in PCR that can drastically influence yield and specificity. Fine-tuning it can enhance the overall performance of the reaction."
This careful consideration of enzyme concentration is essential for maximizing the accuracy and efficiency of PCR reactions, making it an integral part of the optimization process. Through systematic adjustments and careful observation, researchers can refine their protocols for improved reproducibility and reliability.
Common PCR Inhibitors
PCR inhibitors play a critical role in the overall success of polymerase chain reaction. Understanding the sources and effects of these inhibitors can greatly enhance the efficiency and reliability of PCR experiments. Inhibitors are substances that interfere with the PCR process, leading to suboptimal results or complete failure of the reaction. Identifying and addressing these inhibitors is essential for any laboratory technician or researcher involved in DNA amplification.
Sources of Contamination
Contamination occurs from various sources and can lead to unwanted PCR inhibitors. Common sources include:
- Environmental contamination: Dust, aerosols, and other particulates present in the laboratory environment can carry nucleases or other inhibitors.
- Biological contamination: Bacterial DNA or unwanted human DNA can be introduced during sample handling or preparation.
- Reagent impurities: Chemicals used in PCR, including enzymes or buffers, may contain inhibitors if not properly purified.
Efforts must be made to minimize risks associated with these sources of contamination. Adopting stringent laboratory practices such as using barrier pipette tips, maintaining a clean workspace, and employing negative controls can prevent unwanted interference during PCR.
Effects of PCR Inhibitors
The effects of PCR inhibitors can vary based on their nature and concentration. Common effects include:
- Reduced amplification efficiency: Inhibitors disrupt the enzyme activity, leading to lower yield of amplified products. This inefficiency can result in faint bands or no observable bands in gel electrophoresis.
- Increased variability: Presence of inhibitors can lead to inconsistent results, affecting reproducibility. Variability can cause difficulty in quantifying target DNA, which is especially crucial for quantitative PCR applications.
- Non-specific amplification: Some inhibitors may promote non-specific binding of primers, resulting in unexpected products. This phenomenon can further complicate data interpretation and conclusions drawn from PCR experiments.
Emerging Technologies in PCR Optimization
The need for efficiency and accuracy in Polymerase Chain Reaction (PCR) has led to the development of various emerging technologies. These innovations play a significant role in optimizing the PCR process, resulting in enhanced performance and reliability. Understanding these advanced techniques is critical for researchers aiming to achieve precise and reproducible results.
One of the primary benefits of integrating emerging technologies in PCR lies in their ability to minimize human error and improve data accuracy. Modern PCR systems often incorporate automated workflows, leading to faster turnaround times and reduced variability between experiments. This automation not only saves time but also enhances overall lab productivity.
Real-Time PCR Advances
Real-time PCR, also known as quantitative PCR (qPCR), represents a profound leap forward in PCR methodology. This technology allows for the monitoring of the PCR reaction in real-time, providing immediate feedback on amplification progress. By employing fluorescent dyes or probes, it quantifies the amount of DNA present at every cycle, enabling the assessment of initial template quantity with high precision.
The real-time aspect of this technique offers several advantages:
- Higher Sensitivity: It can detect low levels of target DNA that traditional methods may miss.
- Quantitative Data: Researchers can obtain quantitative results that are crucial for experiments requiring precise measurements.
- Streamlined Process: The ability to monitor reactions in real-time reduces the need for subsequent gel electrophoresis, making experiments more efficient.
Incorporating real-time PCR into workflows can significantly elevate the accuracy and reliability of results, solidifying its place in genomic research and diagnostics.
Digital PCR and Its Advantages
Digital PCR (dPCR) introduces a paradigm shift by providing an absolute measure of nucleic acid quantities without the need for standard curves. This technology partitions the PCR reaction into thousands or millions of individual micro-reactions, allowing for precise quantification of target DNA.
Some advantages of digital PCR include:
- Absolute Quantification: It eliminates ambiguities associated with relative quantification methods.
- Increased Specificity: dPCR is less affected by inhibitors, making it reliable in challenging samples.
- Flexibility: It can be used for various applications, including rare variant detection and copy number variation analysis.
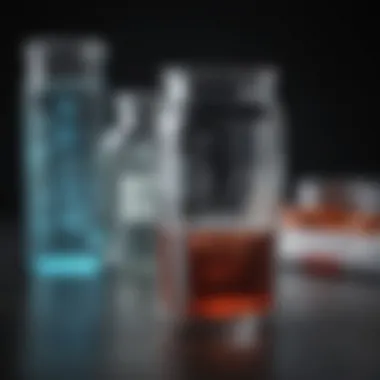
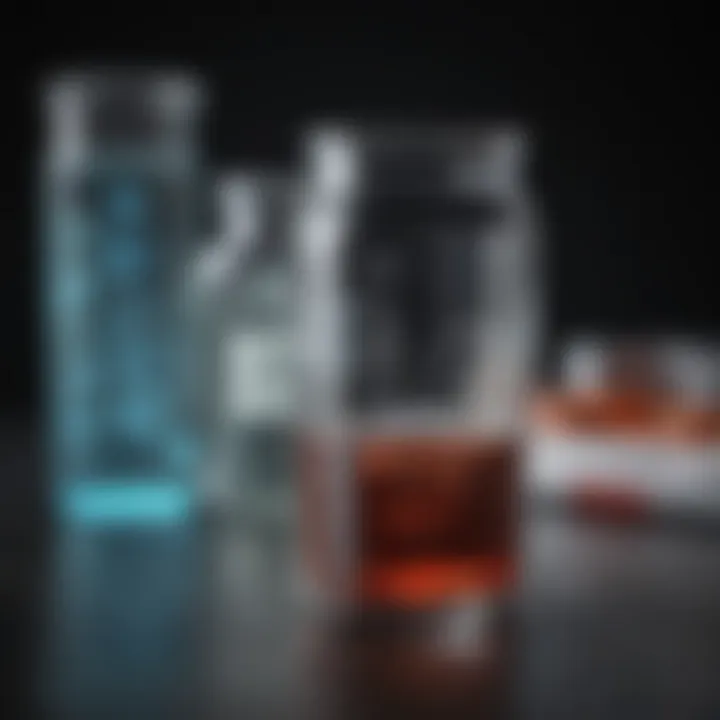
The precision and reliability of digital PCR position it as an indispensable tool for researchers needing to navigate complex genetic landscapes.
"Emerging technologies like real-time and digital PCR stand at the forefront of scientific advancement, paving the way for groundbreaking discoveries in molecular biology."
Best Practices for PCR Optimization
The main aim of PCR optimization is to enhance the reliability of amplification results. By following best practices, researchers can standardize their methods, which can lead to consistent outcomes. This section outlines significant elements that play a role in achieving successful PCRs, while also highlighting several benefits and considerations tied to each practice.
Standardization of Protocols
Standardizing PCR protocols is critical for reproducibility. When researchers use the same conditions in their experiments, they can compare results with confidence. This consistency is especially important in collaborative research or multi-laboratory studies. It minimizes variations that might arise from different reagent batches or thermal cycler settings.
Elements to consider in protocol standardization include:
- Reagent Concentrations: Ensure that the concentrations of DNA, primers, and enzymes are consistent across experiments.
- Thermal Cycling Conditions: Document each cycle step, including annealing and extension times. This helps maintain the same thermal profile, preventing discrepancies in amplification.
- Equipment Settings: Specify the make and model of the thermal cycler used. Different machines may have subtle differences in performance, which can affect results.
One of the benefits of standardizing protocols is the simplicity it brings to troubleshooting. If a reaction fails, checking a consistent protocol allows for easier identification of the issue.
Documentation and Data Analysis
Proper documentation is key in any scientific endeavor, including PCR optimization. Keeping detailed records enhances the quality of research and can lead to better understanding and improvement of protocols.
Consider including:
- Experiment Logs: Record all conditions used in each experiment, from reagent lots to environmental factors. This aids in tracking variables that may influence results.
- Data Analysis Protocols: Establish clear guidelines for how results are analyzed. Use consistent methods, such as gel electrophoresis or software tools, for quantifying PCR products.
Data analysis should focus on key performance indicators such as:
- Amplification Efficiency: Measure how well the reactions work under standardized conditions.
- Specificity Testing: Determine the presence of non-specific products to ensure robustness in primer design.
- Reproducibility Metrics: Analyze results across multiple runs to quantify variability.
Clear documentation and thorough data analysis enhance the ability to fine-tune protocols over time, which will contribute directly to improved efficiency and accuracy in experiments.
Summary: Best practices like standardization and meticulous documentation are essential in PCR optimization. They enhance reproducibility and accuracy, leading to a more reliable understanding of experimental results.
Case Studies in PCR Optimization
In the realm of molecular biology, practical insights derived from real-world applications are invaluable. Case studies in PCR optimization offer a rich understanding of the methodologies, challenges, and successes that can arise in the amplification of DNA. By examining specific instances, researchers can appreciate the nuanced impacts of optimization strategies, leading to more effective practices in their own experiments.
The benefits of studying these cases are manifold. They showcase innovative approaches that have been applied in diverse settings, such as clinical diagnostics, forensic analysis, and genetic research. Moreover, they highlight the critical role that optimization plays in achieving reproducibility and accuracy, which are paramount in scientific endeavors. The findings from these case studies often inform guidelines, inspire further investigations, and refine existing protocols.
Successful Applications in Research
Successful applications of PCR optimization serve as guiding lights for researchers. Each application underscores the importance of customizing protocols to meet specific experimental needs.
- Clinical Diagnostics: A notable case involved optimizing PCR protocols for detecting viral infections. Researchers adjusted the primer concentrations and thermal cycling parameters, ultimately enhancing sensitivity and specificity. This optimization resulted in faster diagnosis, improving patient care significantly.
- Forensic Analysis: In forensic science, case studies illustrating the optimization of PCR for low-template DNA samples have transformed the field. Techniques such as increased enzyme concentration and modified annealing temperatures helped overcome limitations. These strategies not only improved yield but also enhanced the robustness of results in criminal investigations.
- Genetic Research: In genetic studies, researchers optimized high-throughput screening for gene expression analysis. Careful adjustments in buffer composition and cycling conditions led to consistent results across multiple experiments, providing a reliable foundation for subsequent genetic explorations.
Further reading on PCR applications can be found at Britannica.
Lessons Learned from Failed PCR
While success stories are inspirational, failures in PCR offer equally critical lessons. Analyzing instances of failed PCR amplifications provides researchers with insights into troubleshooting and refinement.
- Common Pitfalls: Many failures can be traced back to issues such as poor primer design, inadequate template quality, or the presence of PCR inhibitors. A deeper understanding of these factors can prevent recurrent mistakes.
- Custom Protocol Adjustments: One case study revealed that adjusting the buffer conditions led to unexpected results in enzyme activity, resulting in a failed amplification. This highlighted the importance of thoroughly evaluating buffer compositions and optimizing each component.
- Learning from Echoes: A detailed review of multiple failures in a laboratory pointed out the significance of maintaining strict reagent quality. Investigators discovered that using degraded samples significantly impacted their outcomes. Implementing strict quality control measures subsequently led to marked improvements in PCR success rates.
“The journey through failed experiments can yield the most profound insights if approached with a critical mindset.”
By systematically analyzing the lessons learned from these failures, researchers can effectively refine their techniques and methods. This commitment to continual improvement is essential in the pursuit of reliable and replicable scientific results. The amalgamation of both successful applications and lessons from failures establishes a comprehensive framework for PCR optimization, fueling advancement in molecular biology.
Future Directions in PCR Technology
The field of Polymerase Chain Reaction (PCR) continually evolves, reflecting advancements in molecular biology and related technologies. Understanding future directions in PCR technology is crucial for researchers, educators, and professionals who aim to enhance their methodologies and outcomes. The significance of this topic lies in its potential to refine DNA amplification techniques, making them more efficient, accurate, and accessible.
Innovations in Molecular Biology Techniques
Recent innovations in molecular biology techniques pave the way for improved PCR practices. One notable advancement includes the development of novel polymerases, which offer enhanced fidelity, speed, and tolerance to inhibitors. These enzymes enable researchers to perform PCR in challenging conditions, such as low template quality and high contamination levels.
Another vital evolution involves microfluidic technologies. These small-scale devices allow for the miniaturization of PCR processes, enabling faster thermal cycling and reduced reagent consumption. Additionally, they improve the precision of temperature control, which is crucial for optimizing reaction conditions. The integration of these technologies can lead to significant cost reductions and increase the feasibility of field testing, particularly in resource-limited environments.
Moreover, new strategies such as isothermal amplification methods exhibit promising potential. Techniques like LAMP (Loop-mediated Isothermal Amplification) provide alternatives to traditional PCR by circumventing the need for thermal cycling. This results in quicker results, making it a valuable tool in time-sensitive applications, such as medical diagnostics.
Integrating AI in PCR Optimization
Artificial Intelligence (AI) stands to revolutionize PCR optimization through its ability to analyze large datasets and predict optimal conditions. AI’s analytical capabilities can assist in designing more effective primers and reaction conditions tailored to specific experiments.
Machine learning algorithms can process historical PCR data, identifying patterns that may not be evident to human researchers. This can lead to optimization of parameters such as annealing temperatures and incubation times, saving valuable experimental resources and time. In addition, predictive modeling can help assess the impact of different variables on PCR outcomes before actual experimentation begins, leading to more informed decision making.
Incorporating AI tools into existing PCR protocols can enhance both throughput and outcome consistency. By refining experimental designs, researchers can ensure more reliable results. As AI and machine learning technologies continue to evolve, their integration into PCR protocols will likely become a routine practice, opening new avenues for discovery.
"Integrating AI into PCR optimization can fundamentally alter how researchers approach amplification, making it faster and more accurate without compromising quality."