Types of Electron Microscopy: A Comprehensive Overview
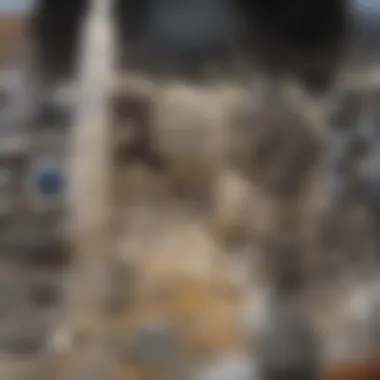
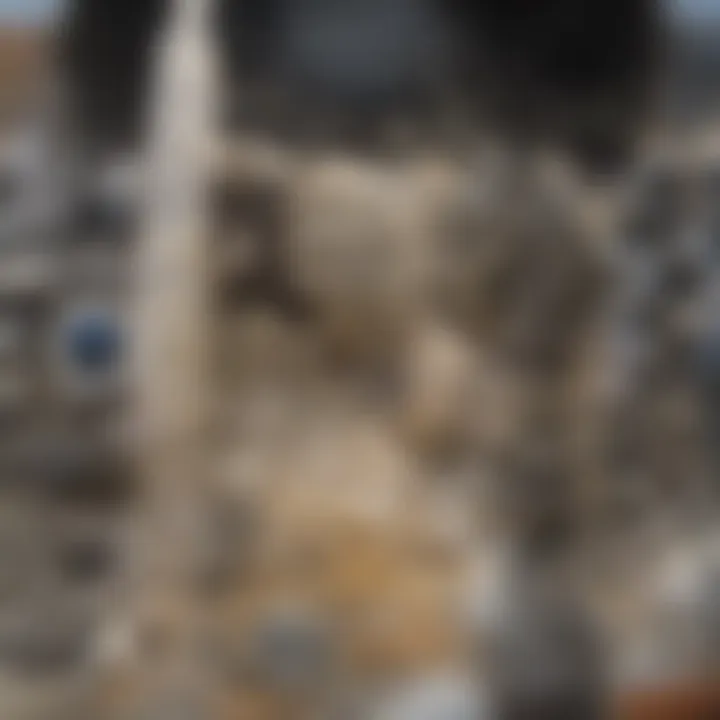
Intro
Electron microscopy, a pivotal advancement in the field of imaging technologies, allows scientists to observe objects at an extraordinary level of detail. Unlike light microscopy, which is limited by the wavelength of visible light, electron microscopy employs electrons, offering a resolution several magnitudes greater. This ability to visualize materials at the atomic and molecular level has revolutionized various scientific disciplines, including biology, materials science, and nanotechnology.
Understanding the types of electron microscopy is essential for researchers and students who aim to utilize these techniques in their studies. Each method comes with unique operational mechanisms and applications, as well as specific limitations. This overview delves into the multifaceted landscape of electron microscopy, shedding light on its distinctive features, how they can be effectively utilized in scientific research, and the potential challenges one might encounter.
The upcoming sections will not only define key terms associated with electron microscopy but also elaborate on the concepts underlying these methods. As we explore the main findings of electron microscopy applications, we will identify areas ripe for future research, indicating that the study of electron microscopy remains a vibrant and evolving field.
Prologue to Electron Microscopy
Electron microscopy serves as a pivotal technique in the exploration of materials and biological specimens at the nanoscale. Its importance stems from the ability to visualize structures that are otherwise inaccessible to traditional optical microscopy methods. With the advent of electron beams and their interaction with matter, researchers can obtain high-resolution images that reveal intricate details of various samples. This article aims to provide a detailed overview of different types of electron microscopy, focusing on their unique characteristics, operational principles, advantages, and limitations.
Historical Context and Development
The evolution of electron microscopy began in the early 20th century when physicists started to explore the wave nature of electrons. The first successful application arose in 1931, when Ernst Ruska and Max Knoll developed the first transmission electron microscope (TEM). This innovation marked a turning point in the study of materials and biological samples, as it allowed scientists to observe structures at a resolution far superior to what was possible with optical microscopes. Over the decades, electron microscopy underwent several advancements, leading to various specialized techniques, such as scanning electron microscopy (SEM) and scanning tunneling microscopy (STM).
From being experimental tools, electron microscopes became indispensable in research laboratories and industrial applications. They have paved the way for significant discoveries in materials science, medicine, and nanotechnology.
Principles of Electron Microscopy
At its core, electron microscopy relies on electron beams rather than visible light to illuminate samples. The principle of electron optics dictates that electrons, akin to light waves, can be manipulated via lenses, allowing for the formation of magnified images. When the electron beam interacts with the specimen, it produces secondary electrons, backscattered electrons, and transmitted electrons, which are detected to form an image.
The resolution achieved by electron microscopy can be well below one nanometer, enabling researchers to observe the fine details of cellular structures or material defects. Two fundamental principles underlie most electron microscopy techniques: wavelength and interaction. The short wavelength of electrons implies a higher resolution capability. The interaction between the electrons and the matter determines the type of image and contrast produced.
In summary, electron microscopy provides an essential window into the fine structure of samples, and it is fundamental for advancing scientific knowledge in various domains. Researchers and students alike benefit from understanding the historical development and the underlying principles, laying a foundation for exploring each microscopy type in subsequent sections. \n
"Understanding electron microscopy is crucial for anyone pursuing research in materials science, biology, and nanotechnology."
As we advance, every technique will shed light on specific applications and challenges within the broader context of electron microscopy.
Transmission Electron Microscopy
Transmission Electron Microscopy (TEM) is a pivotal technique in the field of electron microscopy, distinguished by its ability to achieve high-resolution imaging and characterization of samples at the atomic level. This method is essential in various research domains, including materials science, biology, and nanotechnology. The importance of TEM arises from its operational principles, which allow scientists to visualize the internal structure of materials without significant chemical alteration. As we explore the nuances of TEM, it is essential to understand its operational mechanisms, diverse applications, and the inherent limitations that researchers must navigate.
Operational Mechanisms
In Transmission Electron Microscopy, a concentrated beam of electrons traverses a thin specimen. The setup typically includes a source of electrons, like a tungsten filament or a field emission gun, a series of magnetic lenses, and a detector. The electrons pass through the sample, with some being transmitted while others are scattered, depending on the sample's density and atomic number. Those electrons that pass through are detected and converted to an image, showcasing the internal details of the sample.
Key elements of the operational mechanism include:
- Sample Preparation: The sample must be extremely thin, usually below 100 nanometers, to allow electrons to pass through with minimal scattering.
- Electron Source: The choice of electron source impacts resolution and imaging quality. Field emission guns provide higher brightness and coherence compared to traditional tungsten sources.
- Lenses and Detectors: Magnetic lenses focus the electron beam, while detectors capture transmitted electrons, often using a CCD camera to create digital images.
This method allows for imaging with resolutions down to the atomic scale, making it invaluable for detailed analysis.
Applications in Research
The applications of Transmission Electron Microscopy are abundant and diverse. In materials science, TEM is instrumental in investigating the microstructure of metals, ceramics, and polymers. In biology, it provides insights into cellular structures, allowing researchers to study organelles such as mitochondria and nuclei in great detail. Some specific applications include:
- Nanomaterials Characterization: Provides detailed information on nanostructures, including size, shape, and morphology.
- Defect Analysis: Identifies and characterizes defects within crystalline structures, critical for understanding material properties.
- Biological Samples: Enables the visualization of viruses, proteins, and cells at unprecedented resolutions.
These applications exemplify the versatility of TEM in advancing scientific understanding across multiple fields.
Limitations and Challenges
Despite its advantages, Transmission Electron Microscopy is not without its limitations. Some challenges faced by researchers include:
- Sample Preparation: Preparing samples thin enough for TEM can alter their original structure, which may lead to artifacts in imaging.
- Cost: TEM equipment can be prohibitively expensive, requiring significant investment in both instrumentation and maintenance.
- Complexity: The operational complexity requires skilled personnel, with extensive training needed to interpret the results accurately.
In summary, while Transmission Electron Microscopy provides unparalleled insights into materials and biological structures, researchers must remain vigilant of its limitations and take careful steps in sample preparation and data analysis. It remains a foundational technology in the pursuit of knowledge across scientific disciplines.
Scanning Electron Microscopy
Scanning Electron Microscopy (SEM) is a critical tool in materials science, biology, and nanotechnology. It makes three-dimensional images from a sample's surface by scanning it with focused electron beams. This method is helpful for understanding material properties and structures at microscopic levels. SEM can provide high-resolution images with depth information, which is vital for developing new materials and analyzing existing ones.
Operational Principles
The operational principles of SEM involve several key steps. First, the electron gun generates a beam of electrons. This beam is focused onto the sample surface using electromagnetic lenses. When the electrons collide with the atoms in the sample, they produce various signals, including secondary electrons, backscattered electrons, and X-rays. These signals are collected and converted into an image, allowing researchers to examine the sample's surface morphology and composition.
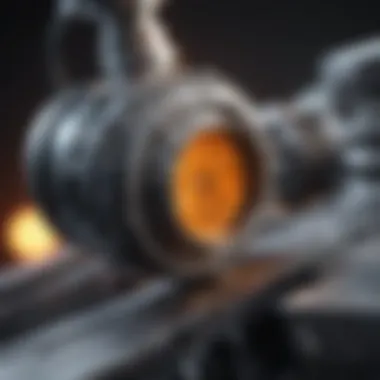
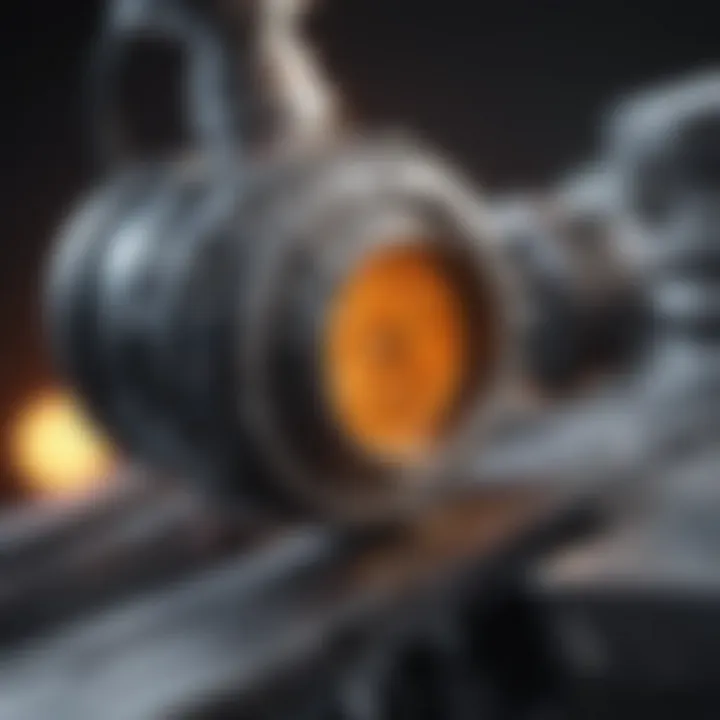
Key points of the process include:
- Electron Beam Generation: Creating electrons through thermionic emission or field emission.
- Sample Interaction: Electrons interacting with the sample's atoms.
- Signal Detection: Collecting the emitted secondary and backscattered electrons.
This imaging technique enables high magnifications, often exceeding 1,000,000 times, allowing observation of fine details such as cracks and surface textures.
Applications in Material Science
SEM plays an essential role in material science by providing insights into various material characteristics. It is commonly used to analyze polymers, metals, ceramics, and composites. Applications include:
- Defect Analysis: Identifying and analyzing defects in materials such as cracks, voids, and inclusions.
- Morphological Studies: Investigating surface morphology, which influences material behaviors.
- Coating Thickness Measurement: Assessing coating (such as paints or chemical layers) thickness and uniformity.
- Nanostructure Design: Helping in the design and development of nano-engineered materials for electronic devices or drug delivery.
Due to its versatility, SEM finds applications in quality control, forensic investigations, and failure analysis.
Challenges and Constraints
While SEM is a powerful imaging tool, it presents several challenges. First, it requires samples to be conductive. Insulating materials can produce charging effects, leading to artifacts in imaging. This can be mitigated with conductive coatings, but that introduces other variables. Additionally, sample preparation can be time-consuming and may alter the original sample if not done carefully.
Other challenges include:
- Cost of Equipment: High costs associated with acquiring and maintaining SEM machines.
- Complex Interpretation: The interpretation of three-dimensional images can be complex due to overlapping signals.
- Vacuum Requirement: The need for a vacuum environment can limit the study of biological samples.
Environmental Scanning Electron Microscopy
Environmental Scanning Electron Microscopy (ESEM) represents a significant advancement in the field of electron microscopy. Its unique capabilities differentiate it from traditional techniques, providing researchers with valuable insights into the nature of materials and biological specimens under conditions closer to their natural environments. This section will explore the distinctive characteristics, versatility in sample analysis, and the challenges associated with ESEM, highlighting its importance in various fields of study.
Unique Features of ESEM
ESEM is notable for its ability to analyze samples in a gaseous environment. Unlike conventional scanning electron microscopy, which operates in a vacuum, ESEM circumvents the need for sample preparation methods that can alter the material's original state. This feature is crucial for observing wet or volatile samples directly, which can be particularly important in fields such as biology and materials science.
The design of ESEM includes a specially designed electron beam column and an environmental chamber, enabling the adjustment of pressure in the sample vicinity. This adaptability allows electron microscopy to expand into realms not previously accessible, such as studying live cells or hydrated materials, without the risk of structural alteration due to desiccation. The ability to visualize samples under real-world conditions opens up new avenues in research and has broad implications for both fundamental science and applied technologies.
Versatility in Sample Types
The capacity of ESEM to handle a variety of sample types is one of its primary advantages. It can accommodate diverse specimens, including:
- Biological Materials: Live cells, tissues, and microorganisms can be studied. The natural environment required for biological processes can be maintained, allowing for authentic observations.
- Hydrated Materials: ESEM can examine materials that contain moisture, such as polymers, gels, and biological samples, without permanent alteration.
- Various States of Matter: This technique can effectively analyze solids, liquids, and even gases. The versatility extends to the study of composite materials, coatings, and thin films.
By accommodating such a range of samples, ESEM broadens the scope of electron microscopy, allowing researchers to investigate phenomena that would be impossible with traditional electron microscopy.
Drawbacks of ESEM
Despite its many benefits, ESEM is not without limitations. Operating at elevated pressures can lead to increased complexity in data interpretation. Here are some of the primary drawbacks associated with ESEM:
- Resolution Limitations: The resolution in ESEM may not match that of conventional SEM techniques, primarily due to scattering effects from gas molecules. This can hinder the ability to resolve fine structural details.
- Cost of Equipment: ESEM systems are generally more expensive than standard SEM equipment. This can pose a barrier for some laboratories.
- Complexity of Operation: The requirement for specific environmental conditions and the need for careful sample handling can increase operational complexity, requiring skilled personnel for effective use.
While ESEM provides an innovative means of examining materials and biological specimens, understanding its limitations is critical for researchers to effectively leverage this technology in their studies.
Cryo-Electron Microscopy
Cryo-Electron Microscopy (Cryo-EM) represents a significant breakthrough in the field of microscopy. This technique captures images of samples at cryogenic temperatures, allowing the observation of biological and materials in near-native states. The ability to visualize macromolecular complexes without the need for crystallization is especially valuable in structural biology.
The principles underlying Cryo-EM involve rapid freezing of samples to prevent the formation of ice crystals that can distort the structure being observed. This preservation technique enables researchers to maintain the original conformation of biomolecules, making it a preferred method for analyzing delicate structures.
Principles of Cryo-EM
The operational fundamentals of Cryo-EM hinge on several key steps. Initially, samples are prepared on a grid and then plunged into liquid ethane, which is kept at -196 degrees Celsius. This quick freezing method avoids the formation of damaging ice crystals, maintaining sample integrity. Once frozen, the sample is loaded into an electron microscope, which utilizes a high-energy electron beam to acquire images.
The imaging process relies on collecting scattered electrons emitted from the sample. Sophisticated algorithms reconstruct 3D models from 2D projections. This allows for detailed visualization of structures at resolutions that were previously unattainable with conventional microscopy.
Application in Structural Biology
Cryo-EM's capacity to generate high-resolution structures is transformative for structural biology. It is particularly effective for studying large, complex proteins that are difficult to crystallize. For instance, Cryo-EM has been instrumental in elucidating the architecture of ribosomes and viruses.
In addition to proteins, Cryo-EM is also applied in understanding lipid bilayers and other membrane proteins. The technique helps scientists analyze the dynamics and alterations of structures during biological processes, thus providing insights into cellular function and disease mechanisms.
Limitations of Cryo-EM
Despite its advantages, Cryo-EM presents certain limitations. The sample preparation process can be technically challenging and requires expertise. Additionally, while Cryo-EM provides excellent structural data, determining the absolute atomic coordinates can be less accurate compared to X-ray crystallography.
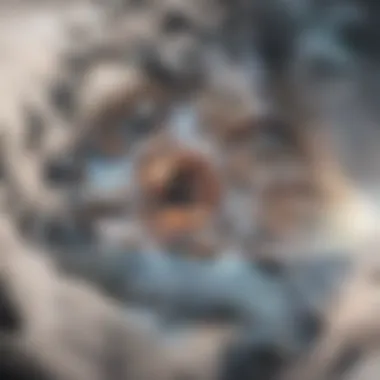
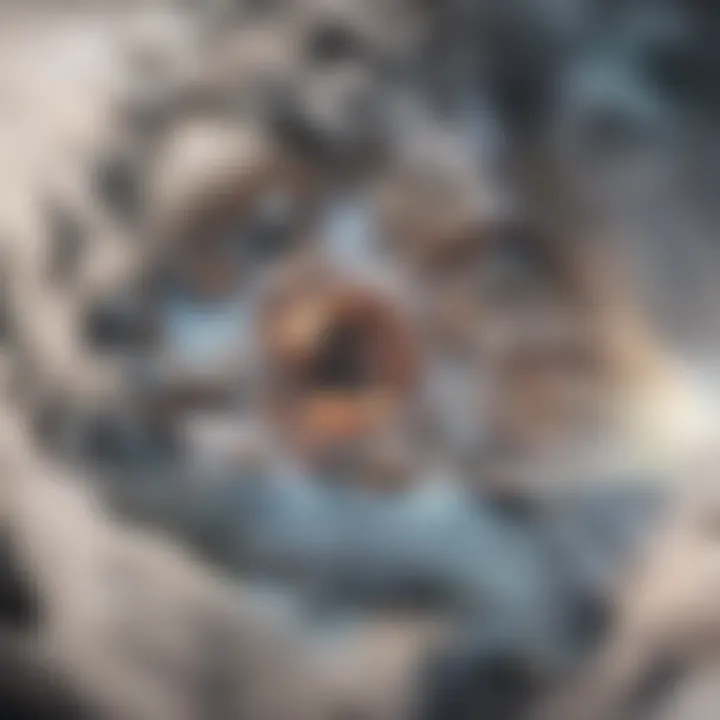
Another challenge lies in the requirement for expensive and sophisticated equipment. Not all research institutions have access to a suitable Cryo-EM system, which can limit its widespread adoption. Furthermore, the data acquisition can be time-consuming, depending on the complexity of the sample being examined.
Focused Ion Beam-Scanning Electron Microscopy
Focused Ion Beam-Scanning Electron Microscopy (FIB-SEM) combines two powerful imaging techniques. This fusion provides a unique perspective on material surfaces and subsurface structures, which is increasingly valuable in research and industrial applications. The ability to generate high-resolution images while also manipulating the sample at the nanoscale elevates FIB-SEM to a significant position within electron microscopy methodologies. Understanding its operational mechanics, applications, and inherent challenges contributes to ongoing advancements in science and technology.
Understanding FIB-SEM
FIB-SEM operates by directing a finely focused beam of ions onto a sample surface, which allows for precise material removal, imaging, and analysis. The scanning electron microscope complements this process by providing high-resolution images of the sample as it is being modified. The ion beam can mill away layers of material to reveal the internal structures of samples. This dual-use nature enables researchers to both visualize and manipulate materials at the nanometer scale.
FIB-SEM is notable for its versatility. It can operate on a variety of materials, including metals, polymers, and semiconductors. The combination of these two techniques allows for comprehensive analysis, combining surface topography and chemical composition in a single workflow. This capability has made FIB-SEM invaluable in many fields, from semiconductor manufacturing to biological research.
Applications in Nanofabrication
The applications of FIB-SEM in nanofabrication are substantial. Researchers utilize this technique for precise patterning and modifying nanoscale structures. The capability to manipulate the material at this scale enables the fabrication of intricate devices. Here are some prominent applications:
- Device Fabrication: FIB-SEM is instrumental in producing microchips by enabling atomically controlled etching processes.
- Sample Preparation: It is used for preparing samples for further electron microscopy analysis, ensuring that the area of interest is adequately exposed and suited for investigation.
- 3D Imaging: Researchers can create three-dimensional reconstructions of complex materials by slicing through layers with the ion beam and imaging each slice with the electron beam.
By facilitating the production of nanoscale devices efficiently, FIB-SEM establishes a platform for innovation in various technological fields, particularly in electronics and nanotechnology.
Challenges of FIB-SEM
Despite its benefits, FIB-SEM faces several challenges that researchers must navigate. Understanding these challenges is critical for optimizing its use. Some of the main difficulties include:
- Material Alteration: The ion beam can induce changes in the material being analyzed, which may alter its properties post-examination.
- Time Consumption: The precision of FIB-SEM can lead to longer operational times, especially if complex structures are to be created or analyzed.
- Cost Considerations: The equipment and maintenance costs for FIB-SEM setups can be substantial, often limiting access to well-funded research facilities.
These challenges must be considered when planning research projects that involve FIB-SEM. The awareness of limitations plays an essential role in maximizing the technique’s effectiveness while ensuring reliable and reproducible results.
"FIB-SEM combines imaging and milling processes, presenting a multifaceted tool for nanotechnology and material science."
Scanning Tunneling Microscopy
Scanning Tunneling Microscopy (STM) is an essential technique in the field of nanotechnology. It provides remarkable insights into the surface structures of various materials at the atomic level. The significance of STM lies not only in its spatial resolution but also in its ability to provide information about the electronic properties of materials.
Operational Mechanism
Understanding the operational mechanism of STM begins with its unique use of quantum tunneling. An STM consists of a sharp metallic tip that approaches very close to the surface to be analyzed, usually within a few nanometers. When the tip is close enough, electrons can tunnel between the tip and the surface. This tunneling current is directly correlated to the distance between the tip and the surface. As the tip scans across the surface, it maintains a constant tunneling current by adjusting its height. This process generates a topographical map of the surface with atomic precision.
Most STM systems operate under ultra-high vacuum and at low temperatures to minimize the thermal effects that can disturb the tunneling process. The images produced are not only topographical but can also reflect the electronic density of states at the surface, providing a profound understanding of the material's electronic behavior.
Important Applications
STM finds numerous applications across various fields, demonstrating its versatility and effectiveness. Some of the key areas include:
- Material Science: Analyzing surface defects and roughness in nanostructured materials.
- Nanotechnology: Fabricating and manipulating nanostructures, enabling the development of nanoscale devices.
- Physics: Studying fundamental phenomena, such as quantum tunneling and electron interaction at the atomic level.
- Biology: Visualizing biological macromolecules and structures with atomic resolution in their natural environment.
The applicability of STM is vast, making it an indispensable tool for researchers looking to analyze materials at the smallest scales.
Limitations of STM
Despite its remarkable capabilities, STM is not without limitations. Several factors affect its usability:
- Surface Requirements: STM works optimally on conductive or semi-conductive surfaces. Non-conductive materials require special preparation or alternate methods.
- Environmental Sensitivity: High sensitivity to vibration and thermal fluctuations requires careful setups to mitigate external noise, which can potentially distort images.
- Limited Field of View: The technique provides detailed atomic-scale images, but the range of view is limited, making it challenging to analyze larger surfaces without multiple scans.
Overall, understanding these limitations is crucial. They should be taken into account when choosing STM as a tool for specific research applications.
"STM has revolutionized surface science by allowing us to visualize and manipulate matter at atomic scale, fundamentally changing our approach towards nanotechnology."
STM remains a powerful technique. Its detailed insights offer essential contributions to ongoing advancements in various scientific domains.
Comparison of Electron Microscopy Types
The comparison of various electron microscopy types is essential in the context of scientific research. It allows researchers to discern the most suitable method for their specific needs. Each type of electron microscopy possesses unique strengths and weaknesses that determine its effectiveness for different applications. This section provides an analytical view that aids in understanding the nuances inherent in each technique.
Strengths and Weaknesses
When analyzing electron microscopy types, it's vital to identify their strengths and weaknesses. For instance, Transmission Electron Microscopy (TEM) excels in offering high-resolution images but requires extensive sample preparation. In contrast, Scanning Electron Microscopy (SEM) provides detailed surface topography with minimal sample preparation but lacks the same level of resolution as TEM.
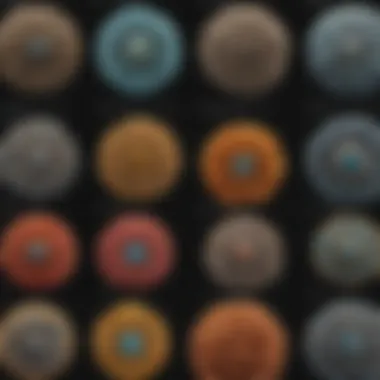
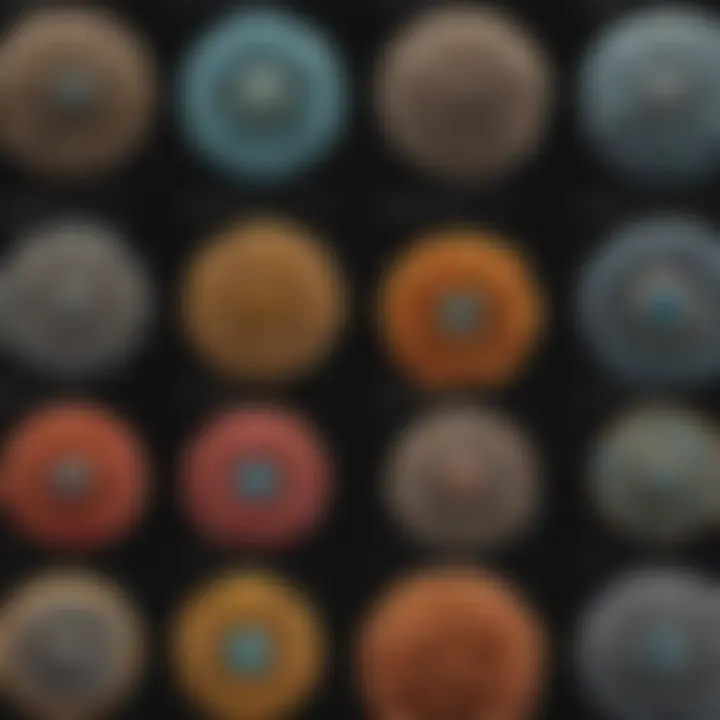
- Strengths of TEM:
- Weaknesses of TEM:
- Strengths of SEM:
- Weaknesses of SEM:
- Exceptional resolution, down to atomic levels
- Capable of analyzing internal structures
- Requires thin samples, limiting applicability
- Time-consuming and technically demanding
- Easy sample preparation
- Provides 3D-like images of surfaces
- Limited information about internal structure
- Lower resolution compared to TEM
This comparative framework aids researchers in making informed decisions based on their specific requirements.
Cost Considerations
Cost is an important factor in choosing an electron microscopy type. Advanced technologies like Cryo-Electron Microscopy (Cryo-EM) and Focused Ion Beam-Scanning Electron Microscopy (FIB-SEM) often come with high operational expenses. These expenses include the cost of equipment purchase, maintenance, and operational consumables, which may not be feasible for all laboratories.
- High-cost techniques:
- Moderate-cost techniques:
- Cryo-EM: High installation and maintenance costs
- FIB-SEM: Requires advanced equipment for precision work
- SEM: Generally more affordable with widespread availability
- ESEM: Still reasonable but may have higher requirements
Researchers must balance the costs with the kind of information needed, ensuring they choose a method that aligns with their budget constraints.
Selecting the Right Type
Selecting the most appropriate electron microscopy type demands a careful assessment of the research goals and constraints. Researchers should consider the nature of their samples along with the type of information they wish to obtain. Different considerations come into play:
- Sample Type: Consider whether the sample is sensitive to electron beams or requires special environments.
- Resolution Needs: Determine the level of detail needed for the research question.
- Budget Constraints: Factor in both initial setup and ongoing costs.
- Technical Expertise: Evaluate the available expertise within the research team, as some techniques are more complex.
It's crucial for researchers to judiciously evaluate these elements to optimize their research outcomes, ensuring that they leverage the most suitable electron microscopy type available to them.
Future Directions in Electron Microscopy
The field of electron microscopy is on the precipice of significant changes. This chapter will explore how advancements in technology and evolving applications are steering this discipline forward. Understanding future directions in electron microscopy is essential for appreciating its growing role in scientific inquiry.
Technological Advancements
One of the main areas of focus in the future of electron microscopy is technological advancements. The development of more sophisticated detectors, like the direct electron detectors, is already setting new standards in imaging quality. These detectors enhance sensitivity and temporal resolution, enabling scientists to observe rapid dynamic processes at the atomic level.
Another noteworthy advancement is the integration of artificial intelligence (AI) and machine learning into electron microscopy workflows. These technologies can analyze vast data sets swiftly, helping researchers identify patterns or irregularities that might otherwise go unnoticed. For instance, AI algorithms can assist in classifying images based on various parameters, improving the efficiency of data processing.
Moreover, multipurpose instruments are gaining attention. Machines that combine different microscopy techniques are being designed. For example, combining scanning transmission electron microscopy (STEM) and scanning electron microscopy (SEM) capabilities into one unit allows researchers to switch between techniques seamlessly, maximizing their analytical potential.
Emerging Applications
Emerging applications of electron microscopy are also contributing to its future. One notable area is in the life sciences. As researchers seek to understand complex biological structures, cryo-electron microscopy is becoming more prominent. This technique allows for the observation of biological samples in their native state, providing insights into protein interactions and cellular dynamics without the artifacts introduced by sample preparation.
In materials science, electron microscopy continues to play a critical role. It enables the analysis of nanoscale materials, contributing to innovations in nanotechnology. For instance, as the demand for stronger, lighter materials increases, electron microscopy helps characterize new alloys and composites at the atomic level, guiding researchers towards improved material properties.
Additionally, the push for sustainability is prompting applications in environmental science. Electron microscopy can analyze pollutants at a molecular level, helping scientists track their sources and effects on ecosystems. This capability plays a crucial role in developing strategies for environmental remediation.
In summary, the future of electron microscopy is marked by rapid technological advancements and exciting new applications. These developments aim not only to improve imaging quality but also to expand the scope of research it can facilitate.
End
The conclusion of the article emphasizes the profound relevance of electron microscopy in modern scientific research. In the discussion, we synthesise insights garnered through exploration of various types and techniques used in electron microscopy. The readers are provided with a comprehensive understanding of the field, highlighting its importance as a tool for advancing knowledge in both materials science and biological research.
Summary of Findings
Through extensive analysis, we have outlined key findings related to the unique types of electron microscopy. Each type has distinct operational methods, suitable applications, and inherent limitations. Transmission Electron Microscopy (TEM) excels in imaging at atomic resolutions, while Scanning Electron Microscopy (SEM) offers excellent surface morphology insights. Environmental Scanning Electron Microscopy (ESEM) provides versatility for wet samples, while Cryo-Electron Microscopy (Cryo-EM) plays a critical role in structural biology. Furthermore, Scanning Tunneling Microscopy (STM) facilitates atomic-scale imaging, and Focused Ion Beam-Scanning Electron Microscopy (FIB-SEM) is essential for nanofabrication.
- Key findings include:
- Each type possesses unique strengths and weaknesses.
- Limitations often include cost, operational complexity, and sample preparation challenges.
- Understanding the differences informs effective selection for specific research needs.
The Importance of Electron Microscopy in Science
Electron microscopy stands as an invaluable asset within the scientific community. Its advances have not only permitted unprecedented resolutions but also opened avenues to investigate phenomena previously beyond reach. With applications spanning across disciplines such as material sciences, biology, and nanotechnology, its contributions shape our understanding of the physical world at microscopic levels.
In scientific study, precise visual representation is crucial. Electron microscopy allows researchers to visualize structures and interactions at scales that are otherwise unattainable. Moreover, its role in discovering novel materials and biological mechanisms remains vital. Overall, the importance of electron microscopy cannot be overstated as it continues to drive innovation and knowledge across various fields.
"Electron microscopy is not just a technique; it is a gateway to understanding the unseen layers of our world."
Future advancements in electron microscopy technologies promise even broader applications and increased accessibility, further solidifying its importance in scientific research. As researchers and educators seek to integrate these methodologies, the implications of enhanced microscopy will undoubtedly echo throughout academia and industry.